+ 0,5
- 0,4
Up Quark
Composition :
Statistics : Generation :
Family :
Interaction forces :
Symbol :
Antiparticle :
Mass :
Decays into :
Electric charge :
Color charge
Spin :
Weak isospin :
Weak hypercharge :
Baryon Number :
Elementary particle
Fermionic
First
Quark
strong, weak,
electromagnetic force
gravity
u
Up Antiquark ( u )
2.2 MeV/c²
stable or
d + e⁺ + νₑ
+⅔ e
Yes
¹/₂
LH : + ¹/₂, RH : 0
LH : + ¹/₃, RH : + ⁴∕₃
1/3
_
UP QUARK
Definition
An up-quark is one of the six fundamental building blocks of matter known as quarks. Quarks are elementary particles that combine in various combinations to form protons, neutrons, and other hadrons, which are the particles that make up the atomic nucleus. The up-quark is a member of the quark family and plays a crucial role in our understanding of the fundamental particles and the strong nuclear force.
Quantum Numbers of the Up-Quark:
-
The up-quark carries a positive electric charge of +2/3e, which is opposite to the -1/3e charge of the down-quark.
-
Quarks also possess other quantum numbers, including color charge. Quarks come in three "colors" - red, green, and blue, which are completely unrelated to actual colors but are a way to describe their strong force interactions. Quarks can interact via the strong nuclear force by exchanging gluons, which carry color charge.
Role in Particle Structure:
-
The up-quark, along with the down-quark, forms the building blocks of nucleons, which are particles found in atomic nuclei. A proton consists of two up-quarks and one down-quark, while a neutron consists of one up-quark and two down-quarks.
-
These combinations of quarks, held together by the exchange of gluons (mediators of the strong force), give rise to the stability of atomic nuclei.
Quantum Field Theory (QFT) and the Strong Force:
-
In the context of quantum field theory, quarks are described as excitations of the quark field. This field permeates all of space and time and is responsible for the creation and annihilation of quarks.
-
The strong force, which is mediated by the exchange of gluons, is described by Quantum Chromodynamics (QCD), a component of the Standard Model. QCD is the theory that governs the behavior of quarks and gluons within hadrons.
-
The strong force is unique in that it becomes stronger as quarks are separated, a phenomenon known as confinement. This explains why quarks are always found in bound states, never as free particles.
Experimental Observations:
-
The existence and properties of up-quarks, as well as other quarks, have been confirmed through experiments conducted in particle accelerators, such as the Large Hadron Collider (LHC).
-
These experiments have provided strong evidence for the Standard Model of particle physics, which incorporates quarks and their interactions.
Combinations with Up-Quarks
Baryons (Spin 1/2):
Proton
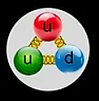
Figure 6 – Proton (uud)
Neutron

Figure 7 – Neutron (udd)
Lambda baryons (Λ) as well as excited states (Λ*)
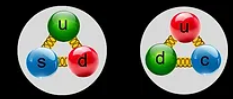
Figure 8 - Lambda Baryon (Λ uds) and (Λc+ udc )
Sigma baryons (Σ+) and (Σ0)
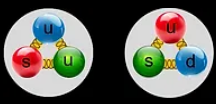
Figure 9 - Sigma Baryons (Σ+ uus), (Σ0 uds)
Xi baryons (Ξ0)
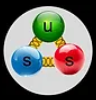
Figure 10 - Xi baryons (Ξ0 uss)
Baryons (Spin 3/2)
Delta baryons (Δ)
To understand the difference between Δ-baryons and protons and neutrons, check the masses and charges tables in the chapter "Baryons".
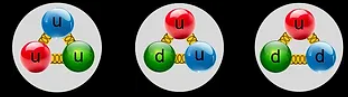
Figure 11 - Delta Barons (Δ++ uuu), (Δ+ uud), (Δ- udd)
Sigama baryons (Σ*)
To understand the difference between Σ*-baryons and (Σ+)/(Σ0) baryons, check the masses and charges tables in the chapter "Baryons".
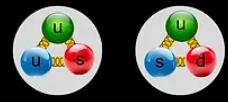
Figure 12 - Σ*-baryon (uus,uds)
Xi baryons (Ξ*)
To understand the difference between Ξ*-baryons and Ξ0 baryons, check the masses and charges tables in the chapter "Baryons".

Figure 13 - Ξ*-baryon (uss)
Pseudoscalar Mesons (Spin 0)
Pions (π+) (π0)
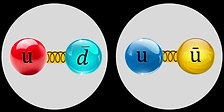
Figure 14 – Pions (π+ u ),(π0 uū)
Kaons (K+)
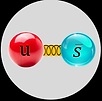
Figure 15 – Kaons (K+ u )
Eta mesons (η) and (η')

Figure 16 - Eta mesons (η) [1]
D mesons

Figure 17 - D mesons
B mesons (B+)
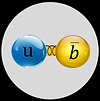
Figure 18 - B mesons (B+)
T mesons
T-mesons are hypothetical mesons. Because of the top quark's short lifetime, T-mesons are not expected to be found in nature.

Figure 19 - T mesons (T+ )
Vector Mesons (Spin 1)
ρ-meson
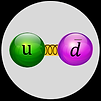
Figure 20 - ρ-meson
K* meson

Figure 21 - K*-meson
ω-meson
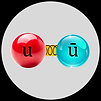
Figure 22 - ω-meson
Creation of Up-Quarks
Up quarks can be produced through a variety of high-energy processes, many of which involve particle accelerators or naturally occurring high-energy events. Here are some ways that up quarks can be created:
Particle Collisions in Accelerators: high-energy particle accelerators, like the Large Hadron Collider (LHC), can speed up particles to near light speed and then collide them. These high-energy collisions can create up quarks along with other particles. For instance, a high-energy collision could turn a gluon (a particle responsible for the strong force that holds quarks together) into an up quark and an up antiquark.
Cosmic Ray Interactions: high-energy cosmic rays striking particles in the Earth's atmosphere can lead to the creation of up quarks and other particles. These cosmic rays can have much higher energies than those achievable in human-made accelerators.
Particle Decay that leads to up-quark creation.
Baryon decays
Classical Beta-decay of a neutron into a proton
One of the most common decays involving an up quark is beta decay, in which a neutron (udd) decays into a proton (uud). This involves one of the down quarks in the neutron changing into an up quark, emitting a W- boson which then decays into an electron and an electron antineutrino.
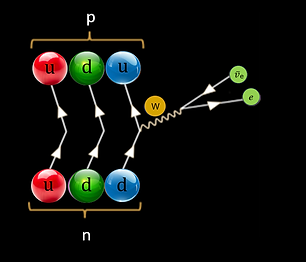
Figure 24 - β⁻ decay of a neutron in a proton
Note that both of these processes are changes of a quark flavour, mediated by the weak interaction, rather than direct decays of the quark itself.
Neutron beta decay plays a fundamental role in nuclear physics, astrophysics, and our understanding of particle interactions. It is a key process in the formation of elements in stars, and the study of beta decay has contributed significantly to our understanding of the weak nuclear force and the electroweak unification, a cornerstone of the Standard Model of particle physics.
Initial State:
In neutron beta decay, the process begins with a neutron, which is a subatomic particle found in the nucleus of atoms. Neutrons are electrically neutral particles and are composed of three quarks (two down quarks and one up quark)
Three Decaying Particles:
Neutron beta decay results in the transformation of the initial neutron into three different particles:
A proton: This is one of the constituents of atomic nuclei, carrying a positive electric charge.
An electron: An elementary particle with a negative electric charge, which orbits the atomic nucleus.
An antineutrino (ν̅e): A nearly massless, electrically neutral particle. Antineutrinos are very difficult to detect due to their weak interactions with matter.
Weak Interaction:
Neutron beta decay is primarily governed by the weak nuclear force, one of the four fundamental forces of nature. The weak force is responsible for the transformation of one type of quark into another and plays a crucial role in the behavior of subatomic particles.
Conservation Laws:
Neutron beta decay conserves several important quantities:
Baryon Number:
The total number of baryons (particles like protons and neutrons) before and after the decay remains the same.
Lepton Number:
The total lepton number (the sum of electrons, muons, and tau particles plus their corresponding neutrinos) is conserved in the process.
Energy and Momentum Conservation:
Energy and momentum are also conserved in accordance with the laws of physics.
Continuous Energy Spectrum:
The energy released in neutron beta decay is not fixed but instead forms a continuous spectrum. This characteristic is a consequence of the three-body decay process, where the proton, electron, and antineutrino share the energy in varying proportions.
Rate of Decay:
The rate at which neutrons undergo beta decay is determined by the properties of the weak force, specifically by the strength of the weak coupling constant, as well as the available phase space for the decay products.
Experimental Confirmation:
Neutron beta decay has been extensively studied and experimentally confirmed. Precise measurements of the decay rate and the energy spectrum of emitted electrons have provided valuable information for testing the predictions of the electroweak theory, which unifies the electromagnetic and weak nuclear forces.
Double β-decay
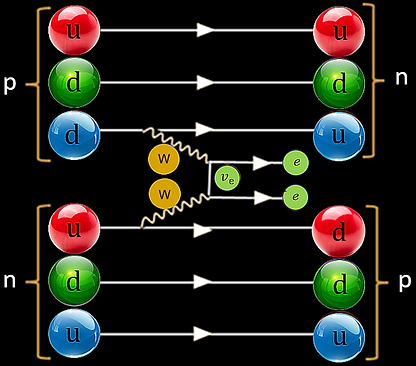
Figure 25 - Two neutrons decay into two protons emitting e and two electrons
Sigma Baryon Decay (Σ-baryon decay)
Certain types of sigma baryons, which contain strange quarks, can decay into other baryons containing up quarks. For instance, a Sigma baryon (Σ⁺) can decay into a neutron (udd) and a pion (π+), resulting in a change from a strange quark to an up quark
or a Σ+ decay into a proton and a π0, resulting in a change from a strange quark to an up quark.

Figure 27 - Sigma-plus (Σ⁺) decay into neutron and pion (π+)
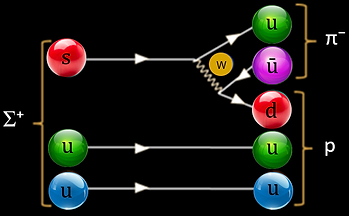
Figure 28 – Sigma (Σ+) decay into proton and pion (π⁻)
Xi-baryon decay
Xi-plus baryon decays into a lambda baryon and a pi-zero meson resulting in a change from a strange quark to an up quark.
Or a Xi-minus baryon decays into a lambda baryon and a pi-minus mason

Figure 29 - Xi (Ξ⁺) decay into Lambda (Λ⁺) en pion (π⁰)
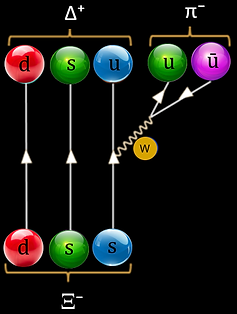
Figure 30 - Xi (Ξ⁻) decay into lambda (Λ⁰) and pion (π⁻)
Pseudoscalar Meson Decay
Kaon Decay (K-meson decay):
Kaons (K-mesons) are particles that contain a strange quark and can decay into particles containing an up quark. For example, a neutral kaon (K⁰) can decay into two pions (π⁺ and π⁻), each of which contains an up quark. The strange quark in the kaon can transform into an up quark in the pions.

Figure 33 - Kaon (K+) decay into 3 pions (π⁻),(π⁺),(π⁺)
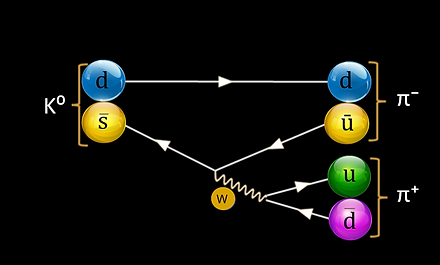
Figure 34 - Decay of Kaon (K0) into 2 pions (π⁻),(π⁺)
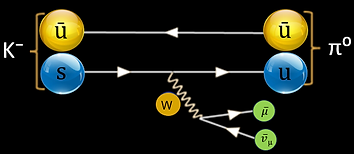
Figure 35 - Kaon (K-) decay into pion (π⁰)
Variations of D⁰ decay
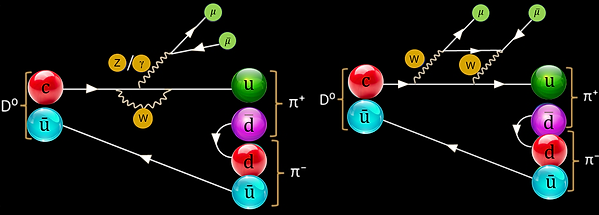
Figure 38 - D0 decay variations into two pions
B⁰ decay

Figure 40 - B0-decay into 3 mesons
Top Quark Decay
In certain processes in high-energy particle physics, top quarks can decay into a W boson and a bottom quark. The W boson can then decay into other particles, including an up-type quark (usually an up or charm quark), leading to the generation of an up quark.
A top quark decay happens after a gluon-gluon fusion :
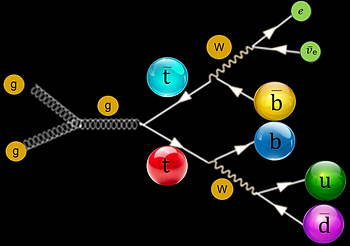
Figure 43 - gluon-gluon fusion with top-quark decay into an up-quark
Big Bang
Right after the Big Bang, the universe was extremely hot and dense, a state of matter known as a quark-gluon plasma. As the universe expanded and cooled, these quarks combined to form protons and neutrons, which are made up of up and down quarks.
Quarks, including up quarks, are always produced in pairs to conserve certain quantum properties. This means when an up quark is created, an up antiquark is also created. It's also important to note that up quarks, like all quarks, are never observed in isolation due to color confinement; they are always found within composite particles like protons and neutrons.
Decay of Up-Quarks
Up quarks are the lightest of all six quark flavors, which limits the possibilities for their decay. Under normal conditions, up quarks do not decay into other quarks because there are no lighter quarks for them to decay into. This is a consequence of the laws of energy conservation - in a decay process, the end products generally have less mass-energy than the initial particle.
However, a decay can happen if the up quark is part of a composite particle such as a proton (uud) or a neutron (udd), as part of certain weak interaction processes:
Proton Decay:
Proton decay is a theoretical concept in particle physics that suggests that protons, one of the stable particles found in atomic nuclei, could eventually decay over an extremely long period of time. This idea is an important prediction arising from certain theories beyond the Standard Model of particle physics.
Theoretical Prediction:
The concept of proton decay arises from certain extensions or theories beyond the Standard Model of particle physics, such as Grand Unified Theories (GUTs). GUTs attempt to unify the fundamental forces of nature (electromagnetism, the weak nuclear force, and the strong nuclear force) into a single, more fundamental force.
Unification of Forces:
In GUTs and similar theories, it is proposed that at extremely high energies (far beyond what we can currently produce in particle accelerators), the forces of electromagnetism and the weak nuclear force merge into a single "electroweak" force. This unification suggests that the particles carrying these forces (the W and Z bosons for the weak force and the photon for electromagnetism) are interconnected.
Proton Decay Mechanism:
The theory of proton decay suggests that, at these incredibly high energies associated with the unification of forces, protons can decay into other particles, such as positrons (antiparticles of electrons) and neutral pions (composite particles made up of quarks and antiquarks). The exact details of the decay mechanism vary depending on the specific GUT or theory considered.
Experimental Searches:
Over several decades, scientists have conducted extensive experiments to detect proton decay. Underground experiments using large detectors filled with water or other materials have been set up to observe potential proton decays but no experimental evidence for proton decay had been conclusively found.
Long Lifetime:
Proton decay, if it does occur, is an extremely rare process with an expected lifetime much longer than the current age of the universe. This makes it challenging to detect, and experiments require vast amounts of time and data collection.
Kaon Decay
Up-quarks can also decay into strange quarks through a process known as kaon decay. This decay involves the weak interaction, where an up-quark transitions to a down-quark or a strange quark. Kaons (K+ and K0) are mesons made up of a quark and an antiquark, and they can be produced in the decays of strange quarks, which can be initiated by the transformation of an up-quark into a down-quark: u → d + W+ → d + K+ (or K0) + π+
Neutrino Interactions
Up-quarks can participate in neutrino interactions where they transform into down-quarks or strange quarks, depending on the flavor of the incoming neutrino. For example, in a process involving an electron neutrino (νe), an up-quark can change into a down-quark: u + νe → d + e-
Top Quark Decay
In rare situations, an up-quark can also be involved in the decay of a top quark (t). In this case, a top quark can decay into a W+ boson and a b-quark. The W+ boson can subsequently decay into various particles, which may involve an up-quark. The exact decay products depend on the specific decay mode of the top quark. This happens e.g. after an gluon-gluon fusion.
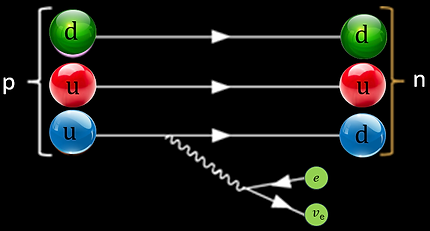
Figure 44 - Proton decay into neutron
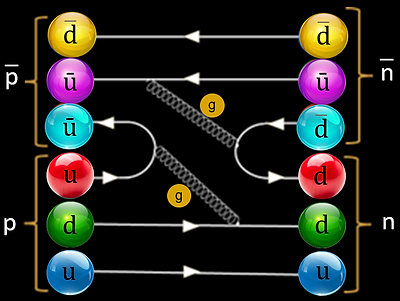
Figure 45 - Proton - Anti-Proton decay into Neutron-Anti-Neutron

Figure 48 - Neutrino interaction u + e → d + e
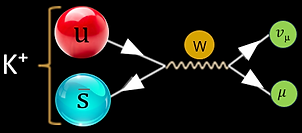
Figure 47 - Up-quark decay in K+ decay
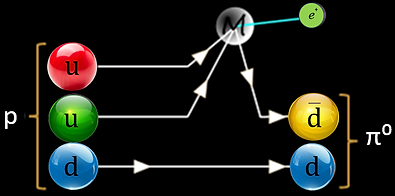
Figure 46 - Monopole catalysis of proton decay through the reaction
p + M → e+ + π⁰ (Rubakov effect)
Annihilation
After the Big Bang, all quantum fields (including quark fields) were massively disturbed, so a lot of particles of all sorts were created
Pair annihilation processes usually result in the production of two photons. However, since quarks are mediated by the strong interaction, they more often result in two gluons (governed by the strong interaction). But, due to quark "confinement" (which essentially means that quarks cannot be observed outside the particles they compose) we can't observe this through the normal scattering experiments we use to observe such phenomena.
However, quarks annihilating into two photons can be observed in processes such as neutral pion decay. The πo is composed of either a down and anti-down quark or a up and anti-up quark. It has been observed that the πo decays into two photons, which means the quark and anti-quark that composed it annihilated!
Also, while not defined as pair annihilation, a quark and antiquark of different types can also interact in a similar way when they interact due to the weak force (which means they are mediated by W- and Z-bosons). These processes can have a variety of outcomes -- two gauge bosons, another quark and anti quark, etc. Note that the pair annihilation process also can result in two gauge bosons, but the type of bosons that can be produced depend on the original particles. For example, a quark and its respective antiquark can annihilate and produce two Z-bosons. But, an up quark and an anti-down quark can annihilate and produce a W+-boson and a Z-boson. The fact that W-bosons have charge is what makes these processes possible. Since W-bosons have charge, they can change quark flavor, as well. So, an up and anti-down quark can interact and produce a down and anti-up quark, if they are mediated by a W--boson.
These types of processes are precisely what were studied at the Tevatron at Fermilab. The Tevatron collided a proton and an anti-proton beam and analyzed the byproducts of the quark-antiquark interactions (the quarks from the protons, antiquarks from the anti-protons) in order to better understand these types of processes.
Nuclear Fusion Processes
Up quarks are fundamental particles that play a crucial role in fusion processes, particularly in the context of nuclear fusion, which occurs in stars like our Sun. Nuclear fusion is the process by which atomic nuclei come together to form a heavier nucleus, releasing a tremendous amount of energy. Up quarks are one of the essential building blocks of protons, which are the primary participants in fusion reactions involving hydrogen nuclei. Here's how up quarks are involved in fusion processes:
Nuclear fusion: A proton is one of the most common particles involved in nuclear fusion. It consists of three quarks: two up quarks (u) and one down quark (d). The combination of two up quarks and one down quark gives the proton its positive electric charge and its stability.
Hydrogen Fusion: The most well-known fusion process in which up quarks are involved is the fusion of hydrogen nuclei (protons) into helium nuclei. This process is the primary source of energy production in stars like the Sun.
Proton-Proton Chain: In the Sun's core, where temperatures and pressures are extremely high, the proton-proton chain is the dominant fusion process. This chain involves several steps, all of which rely on the interactions of protons (composed of up and down quarks):
Step 1 - Formation of Deuterium: Two protons come close together, and one of them undergoes a weak interaction, converting one of its up quarks into a down quark. This results in the formation of a neutron and a proton. The neutron and proton then combine to form deuterium, which is a nucleus consisting of one proton and one neutron.
Step 2 - Formation of Helium-3: Another proton collides with the deuterium nucleus, resulting in the creation of helium-3, which contains two protons and one neutron.
Step 3 - Formation of Helium-4: Two helium-3 nuclei collide and fuse to form helium-4, consisting of two protons and two neutrons. In this step, two protons are released as byproducts.
Energy Release: The fusion of four protons into one helium-4 nucleus, as described in the proton-proton chain, is accompanied by the release of a substantial amount of energy. This energy is emitted in the form of gamma-ray photons, which eventually get converted into heat and light as they move from the Sun's core to its surface.
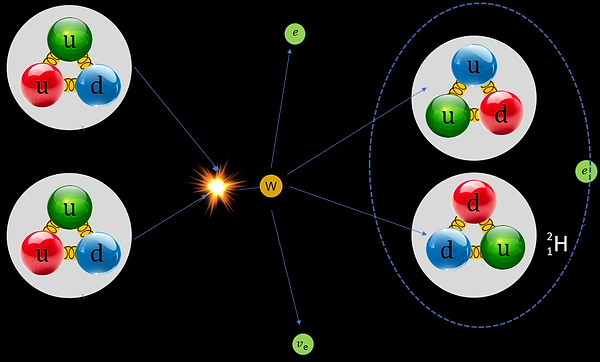
Figure 52 - Formation of Helium 3 (3He)

Figure 51 - Formation of Deuterium
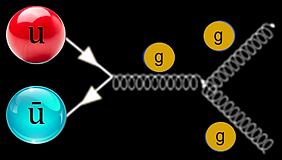
Figure 49 - Up Quark Annihilation resulting in gluons

Figure 50 - Up Quark annihilation resulting in an electron and a positron

Figure 53 - Formation of Helium-4 (4He)
Literature
-
Light Quarks at Large N. Daniel Davies, Michael Dine, and Benjamin V. Lehmann (2022)
-
On the generation of the quarks through spontaneous symmetry breaking. Voicu Dolocan. Faculty of Physics, University of Bucharest, Bucharest, Romania
-
A. Ali, G. Kramer; Kramer (2011). "JETS and QCD: A historical review of the discovery of the quark and gluon jets and its impact on QCD". European Physical Journal H. 36 (2): 245. arXiv:1012.2288. Bibcode:2011EPJH...36..245A. doi:10.1140/epjh/e2011-10047-1. S2CID 54062126.
-
R. Nave. "Quarks". HyperPhysics. Georgia State University, Department of Physics and Astronomy. Retrieved 2008-06-29.
-
A. Pickering (1984). Constructing Quarks. University of Chicago Press. pp. 114–125. ISBN 978-0-226-66799-7.
-
Ambiguities in the Up-Quark Mass. Michael Creutz. Brookhaven National Laboratory (2004)
-
Ruling Out the Massless Up-Quark Solution to the Strong CP Problem by Computing the Topological Mass Contribution with Lattice QCD. Constantia Alexandrou, Jacob Finkenrath, Lena Funcke, Karl Jansen, Bartosz Kostrzewa, Ferenc Pittler and Carsten Urbach (2021)
-
The theory of quark confinement. V. N. Gribov (Landau Institute for Theoretical Physics, Moscow) (1999)
-
Up Quark Masses from Down Quark Masses. I. Masina and C.A. Savoy (2006)
-
Precise Calculation of the Up and Down Quark Mass Using an Adjusted Compton Wavelength Common Factor Analysis. Brian DN (University of Wisconsin-Platteville 1 University Plaza, Platteville WI 53818, USA) (2015)
References
[1] Both the pion (π0) and the eta meson (η) are mesons, which means they are composite particles made of a quark and an antiquark. However, they have different properties, even when they share the same quark content in certain states.
Quark Content:
The π0 is a superposition of two states: an up quark and an antiup quark (uu̅), and a down quark and an antidown quark (dd̅).
The η meson is a more complex superposition of states, involving up, down, and strange quarks and their corresponding antiquarks: (uu̅ + dd̅ - 2ss̅) / sqrt(3).
Spin and Parity:
Both the π0 and the η meson have a spin of 0 and a parity of -1 (P=-1), i.e., they are pseudoscalar mesons.
Mass:
The π0 is much lighter than the η meson. As of my knowledge cutoff in September 2021, the mass of the π0 is about 135 MeV/c².
The η meson's mass is about 548 MeV/c².
Lifetime and Decay Modes:
The π0 primarily decays into two photons. Its mean lifetime is about 8.4 x 10^-17 seconds.
The η meson has a longer lifetime and more decay modes, including decay into three pions, a photon and a neutral rho meson, or two photons. Its mean lifetime is about 5 x 10^-19 seconds.
Isospin:
The π0 has an isospin of 1, meaning it is part of an isospin triplet with π+ and π-.
The η meson has an isospin of 0, meaning it is a singlet and does not have partners with different charge.
So, while π0 and η can have similar quark content in some states, they differ in terms of their quantum numbers, mass, lifetime, and decay modes. This difference is due to the internal quark structure and dynamics, including the effects of quantum chromodynamics, the theory of the strong interaction.